Breakthrough-yielding laboratory experiments are pursued in parallel with a foundation of theoretical work that informs and guides them. Theory can suggest promising pathways and indicate that others might be dead ends. Professor Chris Van de Walle, who works in computational material science, has spent his thirteen years at UCSB laying the theoretical track to deliver such scientific payloads at the SSLEEC.
Van de Walle’s work requires “figuring out the properties of materials for electrical and opto-electrical applications. We are doing that at the most basic level, the level of atoms,” he says. His group has performed calculations required to solve problems related to various semiconductor materials, including gallium nitride (GaN), the common element in a wide range of SSLEEC innovations.
“In my group, we don’t do experiments or make devices,” Van de Walle says. “We try to put fundamental knowledge in place to help other people make better materials and devices. We basically solve the quantum-mechanical problem of how electrons and atoms behave in a solid, and we are able to calculate materials properties from first principles.”
As an example, one problem with LEDs is that they are very small and may be only 30-percent efficient. That’s high compared to other forms of light, and SSLEEC researcher Shuji Nakamura achieved an LED with 50-percent efficiency in 2016 (and a blue LED is now 84-percent efficient), but it is still not nearly 100 percent. That’s a problem because the energy that is not emitted as light is released as heat, and because the semiconductor is so small, it doesn’t have enough surface area to carry the heat away, so a heat sink has to be attached to the bottom of the bulb, making it bigger and more expensive.
Figuring out why the LED has limited efficiency is therefore important, but, according to Van de Walle, “It’s difficult to establish, experimentally, what goes on inside an LED.” So around 2007, he began calculating what happens to the electrons and holes that are injected into the semiconductor material to produce light. The majority of them should combine and generate light, but, given the limited efficiency, some of them had to be doing something else. He wondered what that was.
One important breakthrough was discovering that a process known as “Auger recombination” can be an important loss mechanism, impeding efficiency in a GaN LED. Sometimes when an electron combines with a hole and a certain amount of energy is generated, instead of being emitted as light, the energy is used by another electron to raise itself to a higher energy state. “That’s a loss mechanism, because it doesn’t result in light being emitted,” Van de Walle explains.
Until that discovery, the conventional wisdom had been that Auger recombination, recognized as a loss mechanism in other semiconductors that emit longer-wavelength red or infrared light would not occur in GaN–based semiconductors emitting shorter-wavelength green and blue light. Calculations performed by Van de Walle’s group showed that assumption to be false, demonstrating not only that Auger recombination also affects the efficiency of green and blue LEDS, but that it becomes increasingly important “as you crank up the current in an LED.”
“That’s a problem,” says Van de Walle, “because when you have an LED, you would like to put in as much current as possible to produce as much light as possible.” His findings made it clear, however, that adding current adds more electrons, that those electrons create Auger recombination, and that as a result, efficiency decreases. That effect had been observed and was referred to as “efficiency droop,” but while it was well studied and well documented as a phenomenon, its dynamics were not well understood.
“Based on our work, we were able to say that Auger recombination causes the droop at the higher current,” Van de Walle says. “People had thought they could just engineer the droop away, but you can’t. The Auger recombination is an intrinsic property of the material, so it cannot be avoided. The only solution is not to run an LED at such high current, and that’s why LED manufacturers now design their LEDS to be used at lower currents.”
Doubters remained, however, even after Van de Walle and his team found that the Auger mechanism was important for short-wavelength LEDs. Van de Walle’s SSLEEC colleagues Jim Speck and Claude Weisbuch put the debate to rest by running an experiment to explicitly detect whether Auger recombination was happening. And, in fact, they were able to detect a signature of the high-energy electrons.
“That was the smoking gun,” Van de Walle recalls. “Auger had to be happening, because if it weren’t, there would be no way you would have electrons at such a high energy state. And the number of those high-energy electrons increased as the current increased. It scaled exactly. Auger was the only explanation.”
Van de Walle has also led research to determine whether zinc oxide (ZnO), a cheap and readily available crystalline semiconductor, could be used as an LED. Because it is transparent, ZnO is already used as a contact in digital displays where an opaque metallic material would not work because no light would come out. The ZnO spreads the current, which gets delivered to the LED. But ZnO has an unusual property: unlike other semiconductors, which have to be doped, by adding other materials, to become conductive, when ZnO is grown, it is spontaneously conductive, even though no impurities have been added. That was puzzling.
Van de Walle proposed that, in growing ZnO, hydrogen was being added and causing the conductivity. Hyrdogen is everywhere and is used in some crystal-growing processes, so, Van de Walle surmised, the fact that it would get incorporated into ZnO and act as the impurity that caused the ZnO to become conductive, was not surprising. But that process had been overlooked because hydrogen does not have that effect on other semiconductors. With silicon, for instance, which is conductive to begin with, hydrogen reduces its conductivity, making its effect on ZnO counterintuitive.
Van de Walle and Anderson Janotti, his co-researcher at the time, figured that the effect resulted from a very unusual bond that hydrogen formed inside the ZnO. They also determined that ZnO could not be an LED itself, even if the unintended conductivity caused by hydrogen were avoided. To get light out of an LED requires injecting current from both sides of the device. On one side are negatively charged electrons; on the other are positively charged holes. When they meet in the middle, they interact, releasing energy as light. But with ZnO, it turned out that the positively charged, p-type, part of the process simply did not work and could not be made to work.
“Based on the nature of zinc oxide as a crystal and the energy states within it, you’re just never going to be able to make it p-type conductive, and if you can’t do that, you can’t make an LED,” Van de Walle adds. “It’s better to know this than to be one of those who are, in my opinion, wasting their time trying to do something that is fundamentally impossible.”
And that is where theory hits the hard, unforgiving road.
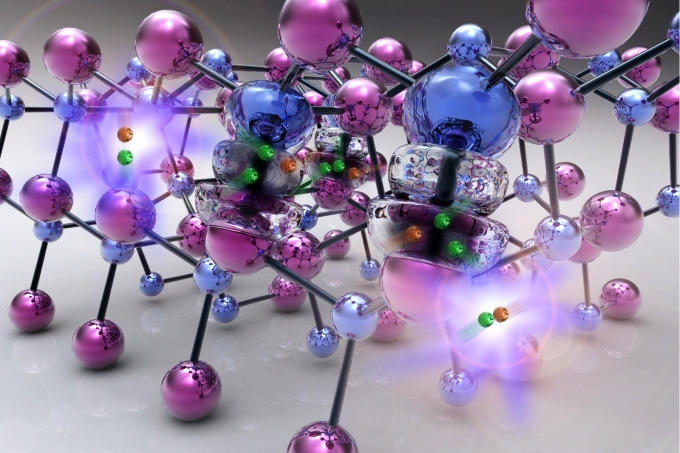
Artist's rendering of radiative recombination