It seems simple enough: a person takes a medicine at a given dose to treat a specific condition. But dosing is not an exact science. Different people, even people who weigh about the same, metabolize medicines at different rates, and dosing based on body mass does not necessarily capture those metabolic differences between patients. Differences between the biology of men and women can further complicate drug dosing.
For some time now, chemistry professor and director of the UCSB Center for Bioengineering, Kevin Plaxco, has been leading the development of electrochemical sensors to conduct real-time monitoring of drug levels in the body. Coupled to feedback control, that could make it possible to monitor the effective drug concentration in a patient’s blood moment to moment and continuously fine-tune the amount of the drug that is released.
To date, Plaxco’s process has been built around a tiny gold-wire sensor that can fit through a very narrow, 22-gauge, hypodermic needle. The wire is coated with a type of DNA molecule, called an “aptamer,” to which the specific drug of interest can stick, or “bind.”
In current experiments, the DNA-coated sensor is then inserted into the body of a rat, where binding of a specific molecule to the aptamer — so far, a half-dozen different drugs, including several “last-line-of-defense antibiotics,” have been administered — generates an easily detectable electronic signal that scales with the concentration of the target molecule. So far, the Plaxco group has adapted multiple sensors to such in-vivo deployment and used them to measure concentrations of multiple drugs in the living body in real time.
A limitation to their approach, however, results from using DNA as the recognition element, because it can recognize only a limited number of molecules. In further experiments, Plaxco and Martin Kurnik, a postdoctoral researcher working in his lab, have sought to adapt protein molecules to the platform, because their much-greater chemical complexity allows them to bind a far greater range of target molecules.
But using proteins presents a problem of its own. Because the gold-plated sensors pick up and provide an electrochemical readout, their surface has to be a charged conductor.
“Under the conditions we employ, the surface of our sensor has a negative charge,” Plaxco says. And, like all DNA molecules, aptamers also have a negative charge. That prevents the aptamers from adhering inappropriately to the sensor surface, i.e. in a way that causes the DNA to unfold, losing its structure and function.
But when a protein — which, unlike a DNA molecule, contains both negative and positive charges — is bonded to an artificial surface, such as the sensor, it stops functioning. “Attaching DNA to a surface does not change its folding stability very much,” Plaxco says. “But when we tried proteins, they just unfolded, stuck on the surface, and lost their function. That's what got us thinking about this problem.”
Plaxco notes that several theories explain the mechanisms by which the protein’s attachment interaction with the surface would change the protein’s stability, but it seems to be closely tied to charge. He believes that when the protein attaches to the sensor, its positive charges are drawn to the negative charge of the gold surface, causing the protein to unfold and cease functioning.
That’s the theory, “But until now,” Plaxco says, “no one has been able to measure that experimentally.”
A paper published in August in the Proceedings of the National Academy of Sciences, co-authored by Kurnik and Plaxco, explains a new procedure for understanding the chemistry and physics of the unfolding process.
Specifically, the method lets researchers measure by how much the relative amounts of folded and unfolded protein molecules (i.e., the protein’s “stability”) differ when a protein is confined to a surface compared to when it is free in solution, and to systematically explore the physical origins of that effect.
“This allows us to start to dissect what drives proteins to unfold on the surface,” Plaxco says. “Our technique gives us great control over the chemistry and the charge on the surface. We can tune those variables and see how changes in the parameters change a protein’s stability.”
“The novelty in this is that we're performing a quantitative measurement of how the folding physics of proteins are affected by their being confined to a surface,” says Kurnik. “As far as we are aware, there are no prior examples of this.
"What I hope is that, first, this will allow us to make protein-based biosensors. That could make the unique sensor platform that Kevin has developed applicable to a much wider range of molecules, allowing it to be used as a diagnostic tool for a broader variety of medical conditions.”
The research may also prove valuable in understanding some fundamentals of cell biology. Kurnik explains: “Because our method makes it easier than ever for researchers to perform high-precision measurements of protein-surface interactions, I hope that it will also be used to provide new insights into the physics of how proteins interact with various surfaces, including ones that mimic the properties of biological membranes.
"Our current understanding of these effects is limited, and we cannot claim to comprehend the molecular biology of the cell without quantitative descriptions of the interplay between a cell’s soluble proteins and its multitude of membrane surfaces.”
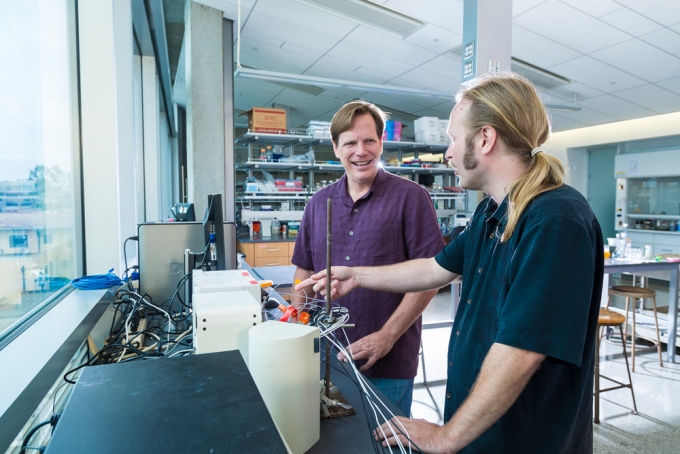
Martin Kurnik (left) and Kevin Plaxco at the bench in the Plaxco lab.